Here are the reports, broken down by topic:
1.
El Nino
Dyna McCirculation:
I
used sea surface height data from the TOPEX/Poseidon satellite
to monitor the progression of the 1997-1998 El Nino in the Pacific
Ocean.

The height
of the sea surface corresponds in part to the temperature of the
water; warmer water is "higher" while colder, denser water is
"lower." In these images, areas of red and white indicate sea
level height well above average (water that's warmer than usual);
purple and violet indicate below-average sea level height (and
colder water).

The April
1997 image shows an area characterized by above-average sea level
height getting sloshed along the equator toward the Americas by
east-blowing winds.
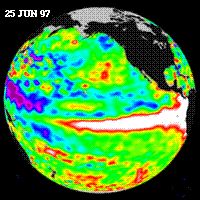
The June 1997
image shows that the warmer (higher sea level) water traveled
across the Pacific and started spreading along the coasts of North
and South America.
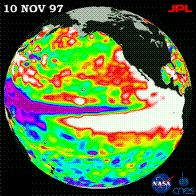
In November
1997, El Nino peaked; sea level reached as high as 35 cm above
average in parts of the eastern Pacific. Above-average sea level
conditions were evident as far north as Alaska and as far south
as Chile.
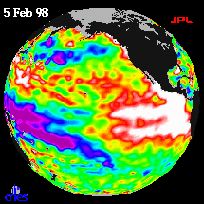
By February
1998, El Nino began to dissipate as the warmer (higher sea level)
water receded toward the equator.
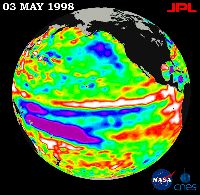
By May 1998,
the eastern Pacific was returning to normal. However, the western
Pacific had not yet returned to normal; the colder (lower sea
level) water, which lingered for several months, affected weather
conditions around the world.
Fisher
Reese: The warm waters that encroach upon the west coast of
the Americas during an El Nino event significantly impact the
food chain, all the way up to humans. Normally, upwelling along
the Pacific coast brings cold water and nutrients to the surface;
the nutrients support a healthy population of phytoplankton, the
tiny ocean "plants" that form the base of the marine food chain.
Two images illustrate cold water upwelling and phytoplankton productivity
along the west coast of the United States: the Advanced Very High
Resolution Radiometer (AVHRR) image shows sea surface temperature,
and the Coastal Zone Color Scanner (CZCS) image indicates phytoplankton
pigment concentration (which corresponds to productivity). In
the AVHRR image, purple and blue denote cold water; in the CZCS
image, red and yellow indicate high phytoplankton production.
Cold water and high productivity typically characterize the Pacific
coast. However, this pattern is disrupted during El Nino, and
fish harvests drop dramatically. Satellites help us better understand
and predict phytoplankton productivity so we can improve how fisheries
are managed.
|
2.
Harmful Algal Blooms
Fisher
Reese: First,
here's some background information to skim before I explain how
I used satellites to study harmful algal blooms. Algal blooms
are natural phenomena that occur when a combination of factors
such as sunlight, nutrients, and water temperature encourage the
growth of one particular species of phytoplankton over others.
Algal blooms are considered harmful if they produce toxins that
adversely affect sea life or humans. For example, Gymnodinium
breve, a common bloom species along the west coast of Florida,
produces a neurotoxin that can contaminate shellfish and make
beachgoers cough. Anoxia (oxygen depletion) can also lead to massive
fish kills associated with harmful algal blooms. Impacts on public
health, local ecology, and tourism underscore the need to study
and monitor harmful algal blooms - and satellites can help.
|
Disadvantages
of Satellite Sensors:
*
Satellite sensors can only "see" the surface of the water.
We can only surmise what is going on below.
*
Only a small percentage of the original light remains after
the long journey from the sun, through the atmosphere, into
the ocean, and back up to the sensor. This means our satellite
instruments and the equations we use to understand sensor
information must be precise so we don't misinterpret the
information.
*
To correctly interpret data from satellite sensors, we must
compare these data to "ground-truth" data. For instance,
before phytoplankton pigment concentration can be derived
from SeaWiFS data, we first have to measure how EM radiation
changes as it interacts with bodies of water with known
quantities of phytoplankton pigment. Then we can develop
models that tell us how to interpret the signals we get
from satellite sensors.
|
|
Algal blooms
are often called "red tides," brown tides," or "yellow tides"
because the abundant phytoplankton actually color the water. Therefore,
satellites that measure ocean color, such as the Sea-viewing Wide
Field Sensor (SeaWiFS) and CZCS (the predecessor of SeaWiFS),
are ideal for detecting and monitoring blooms. This 1978 image
of a G. breve bloom off the southwest coast of Florida is one
of the first ever obtained by CZCS; this image is an "oldie" but
a "goodie." The red, orange, and yellow areas indicate high phytoplankton
concentration. Using satellite data, I can determine the concentration
of the phytoplankton and measure the extent of a bloom, which
are tough to measure from a ship. However, I still need to make
initial measurements on land to confirm what the satellites are
telling me.
View the image
here.
Sally Nitty:
I can use satellite sensors to detect chemical markers that signal
the end of an algal bloom. Phytoplankton populations can decline
for several reasons including: the cells run out of nutrients,
zooplankton eat them, sunlight diminishes, or water temperature
changes. As phytoplankton die, their cell structure breaks down;
"dead materials" are released that give us different signals than
live phytoplankton do. The old CZCS sensor was not sensitive enough
to make a distinction between these different signals. Fortunately,
the new SeaWiFS sensor measures light at the wavelengths that
do reveal the difference so we don't overestimate productivity
and misinterpret the signals.
3.
Storms and Sea Level Rise
Dyna McCirculation:
Satellite sensors are lifesavers when it comes to hurricanes.
Sensors help us to determine the strength and speed of a hurricane
and, more importantly, to make predictions about the path of a
hurricane. With advance notice, people who live in the path of
a storm can protect themselves and their property, and if necessary,
evacuate to a safe location.
View the image
here.
In August 1992, Hurricane Andrew ripped through the Bahamas, Florida,
and Louisiana. It was one of the most destructive hurricanes in
United States history, not to mention the most expensive on record.
Damages in the United States exceeded $25 billion. Considering
the destructive force of Andrew, the direct loss of life was remarkably
low: three in the Bahamas, and 23 died in the United States. The
combination of preparations and evacuations minimized the death
toll.
In 1954, before Doppler Radar and satellite storm tracking systems,
a hurricane similar to Andrew hit the United States with far more
deadly results. On October 15, 1954, the weather forecast for
the Carolinas called for strong winds and rain in the morning
that were to clear in the afternoon. Instead, Hazel, a Category
4 storm like Andrew, made landfall at Myrtle Beach, South Carolina
and caught residents off guard. By the time Hazel fizzled out
in Canada, it had killed around 350 people. So remember, those
spectacular images of hurricanes that you see on the evening news
are more than pretty pictures. They can be lifesavers.
4.
Natural Disasters
Sally Nitty:
Oil
spills might be considered more of an "unnatural" disaster, but
their impact on the environment is real nevertheless. Surprisingly,
major spill events make up just a small percentage of oil pollution
overall, but they can be devastating. Satellite imagery is often
indispensable in the case of major ocean oil spills such as tanker
accidents; we use it to measure the extent of a spill and predict
its movement.
This Synthetic Aperture Radar (SAR) image shows an oil spill along
the coast of Portugal. The image was taken two days after the
Panamanian oil tanker, Cercal, hit a rock and spilled about 1,000
tons of crude oil into the Oporto harbor. The oil quickly spread
offshore. The city of Oporto is shown as a cluster of white dots
on the coast; that's where the spill began. The oil appears much
darker because oil is slick and scatters back less light than
the rough sea surface. Sensors that use wavelengths other than
radar can also detect oil slicks. However, in this case the SAR
instrument was ideal because it operates well in the rainy, foggy
weather that characterized the day of the oil spill. Its radar
waves pass through clouds uninterrupted, unlike visible or infrared
waves.
Sandy Bottoms:
I used three Landsat images to study the effects of the eruption
of Mount St. Helens in southwest Washington on May 18, 1980. View
the images here.
The first
image shows Mount St. Helens about seven years before the eruption.
The white area of the image signifies snow and ice surrounding
the mouth of the volcano. The rest of the image is red, which
indicates forest vegetation.
The next image,
taken a few years after the eruption, shows the extent of the
devastation. The top 1,300 feet of the once mile-high mountain
was lost in the landslide of rock and debris. The grayish-blue
areas represent more than 150 square miles of devastated forest
that are now mud and ash.
The final
image, obtained about a dozen years after the eruption, shows
the forest gradually making a rebound (light red).
5.
Coral Reefs
Sandy Bottoms:
Coral
reefs are typically found in shallow, clear water. They can often
be viewed from space with sensors that use visible and near infrared
wavelengths.
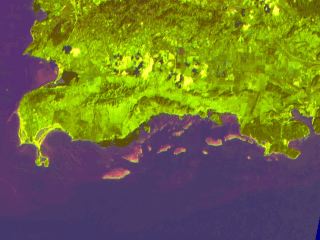
This Landsat
image shows the southwest portion of Puerto Rico. The offshore
reefs are clearly visible (shown in pink/green). We can use satellite
images to map reefs, monitor changes in sea-bottom habitats, and
look for signs of disease or trauma in the reef community.
Fisher
Reese: Many of the organisms that live on a reef fluoresce;
that is, they absorb light and radiate it back out at a longer
wavelength. In the following photographs, various reef creatures
fluoresce particular colors (visible light) after exposure to
a flash of UV light (shorter wavelength than visible light).
View the images
here.
The color
varies depending upon the organism, allowing scientists to identify
species of coral and other reef organisms based upon their fluorescence
signature. A fluorescence sensor mounted on an unmanned underwater
vehicle identifies species and creates bottom maps far more quickly
and comprehensively than divers could survey the area. With a
little more ground work, we may soon be able to use satellites
to map larger areas, which would enhance our ability to monitor
reef health and study species dynamics.
Conclusion:
These reports
highlight just a few of the amazing capabilities of satellite
sensors. There are significant advantages to using satellites
for ocean research; the two most important are repeatability and
coverage. A satellite sensor can regularly repeat its measurements
day-in and day-out for many years. The sensor's ability to track
information over long periods allows us to assemble time series
data to answer questions about weather patterns, productivity
cycles, pollution, and climate change. Like a child's growth chart,
time series data help us see patterns, make predictions, and develop
long-term plans. Satellites also enable us to measure large areas
over short periods of time. Some satellites can cover an entire
ocean in less than an hour, or even the entire globe in just one
day! In contrast, we could send out thousands of ships at once,
and many days later would still have only sampled a tiny portion
of the oceans. Thanks to satellites, our "eyes in the sky," we
have a much clearer picture of the workings of the water world
we are so fortunate to call home.
|